The project
LRC is a project funded by the European Union within the Horizon 2020 research and innovation programme with the objective to push optical spectroscopy frontiers to the intriguing SuperHeavy Elements (SHEs), elements neither occurring in nature nor can be synthesized in minuscule amounts, and about which hardly anything is known. We want to understand how these elements can exist and how the atoms and their nuclei work.
Superheavy Elements
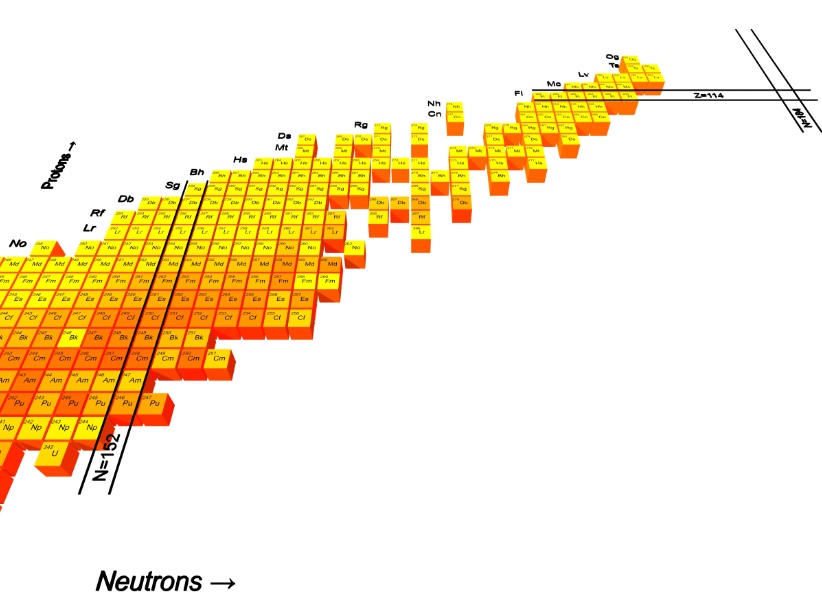
There is always a generic value on the development of understanding about SHEs,
in particular when “new” elements are discovered, because these provide a
fertile ground for testing the borderlines of nuclear existence.
In fact, these elements exhibit an atomic nucleus with so many protons that they
“classically” would not exist at all, due to strong Coulomb repulsion forces
between the protons. However, nuclear shell models do not predict only the SHEs
that we can produce in nuclear fusion-evaporation reactions but also a fabled “island” of nuclear
stability of SHEs at extremely large proton and neutron numbers (north east of the
nuclear map), which is out of reach of current SHE production technologies.
Those elements we know are short-lived and vanish within seconds of their production. If,
however, neutron-rich SHEs from this island can exist and are being produced in
the universe by, e.g., the rapid neutron capture (r-) process, their evidence may
be present in spectra of potential astrophysical sites, like in neutron star mergers.
But then, how to detect them if not by telltale photons from such distant sources,
because they are not primordial?
Hence, established spectral lines from pioneering spectroscopy experiments may serve like fingerprints for multi-messenger
astronomy searching for SHEs from r-process events, which may push
the limits of nuclear stability far beyond the nowadays-achievable.
Moreover, the experimental exploration of atomic emission spectra is essential to
advance our understanding of the atom’s structure.
This is because relativistic, many-body, and quantum-electrodynamic effects become
increasingly important with proton number.
The investigations are also driven by the need for mapping single particle
and collective properties of atomic nuclei, which owe their existence to nuclear
shell effects, in a region of the map where very little or no nuclear spectroscopic
information is known. Given the atomic physics is well understood, measurements of
hyperfine structure and isotope shifts of spectral lines will enable these nuclear
properties (such as spins and moments) to be extracted independent of nuclear model
assumptions and thus will help to improve predictions for next spherical
nuclear-shell closures around the “island of stability”.
Optical Spectroscopy Frontiers
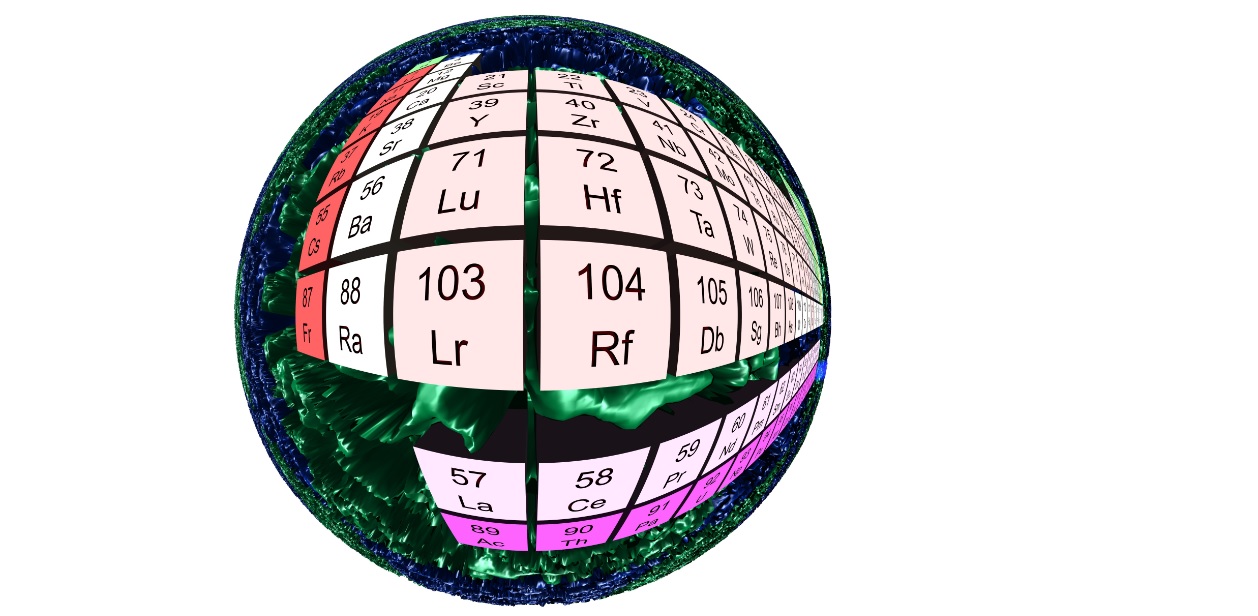
Currently, optical spectroscopy ends at element nobelium (Z=102). Beyond nobelium, the atomic structure is
experimentally unknown and the experiments become extremely challenging.
Any spectroscopy of these superheavy elements would require an extensive search for expected optical
transitions within a broad spectral range inferred from atomic model calculations and the application of
ultra-sensitive methods, capable to measure a single atom at a time.
Contemporary methods based on detecting fluorescent atoms lack sensitivity and thus are not suited for such
studies.
The technique employed in the nobelium studies exploits resonant atomic excitation and ionization with
subsequent radioactive-decay detection. It turned out to be extremely sensitive but also prone to
physicochemical properties of the sample atoms, which may hamper its applicability to the refractory elements
that lay ahead.
To enable such studies beyond nobelium, new developments towards ultra-sensitive spectroscopy approaches
of the next generation in conjunction with high-accuracy model calculations have to be pursued hand in hand.
The LRC approach
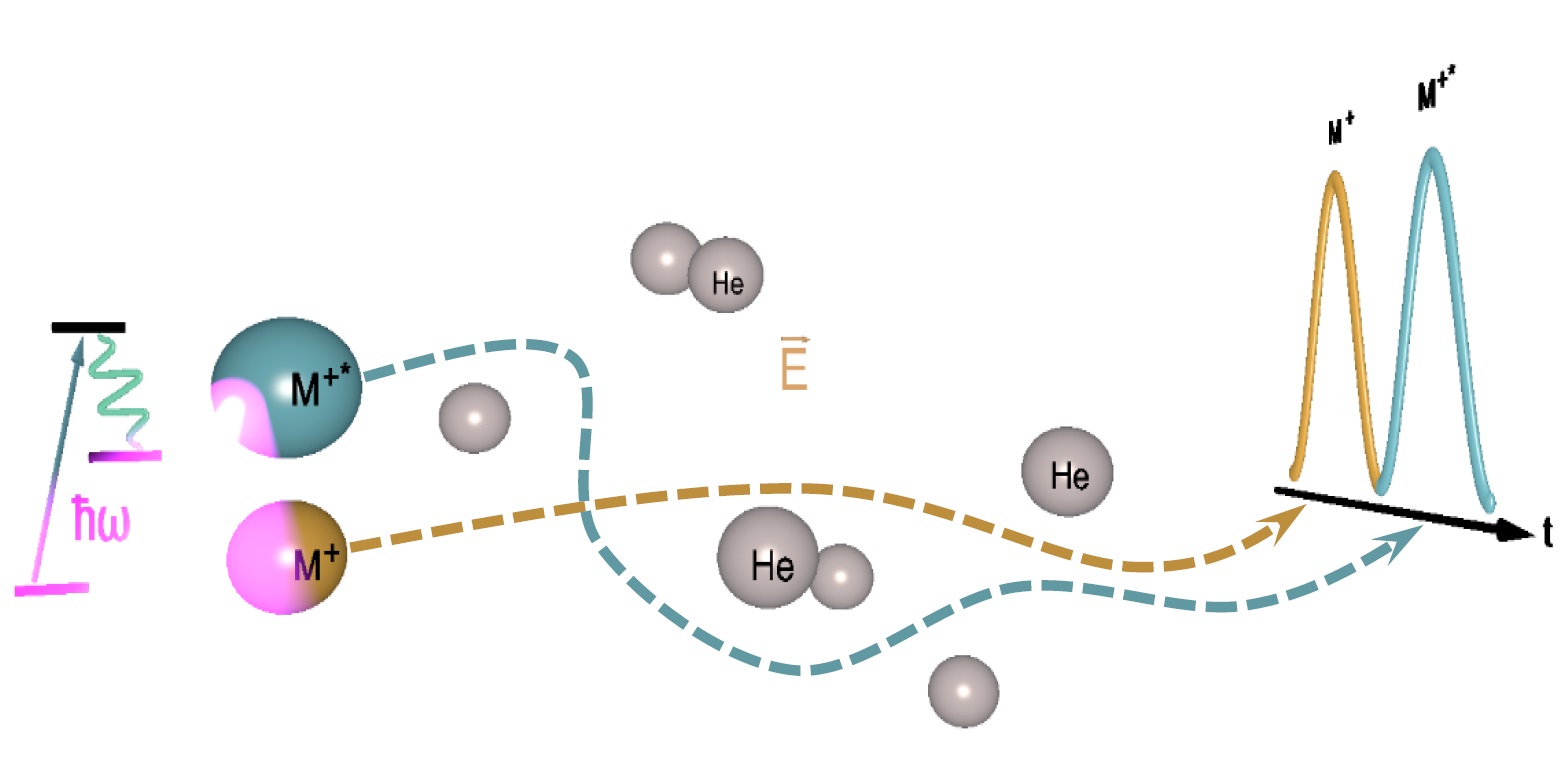
Our way of spectroscopy merges the benefits of a high sensitivity - like in resonance ionization-based
techniques - with the “simplicity” of optical probing as in fluorescence spectroscopy.
We anticipate laser probing of the fusion product ions as delivered from gas catchers behind in-flight
separators and exploit the ion transport properties for the detection of resonant optical pumping.
The investigations in superheavy elements are unique and out of reach for existing experiments
worldwide. Starting with singly-charged lawrencium (Lr, Z=103), available with a modest production yield,
we will search for the ^1S_0-^3P_1 ground-state transition in ^{255}Lr+ to initiate optical pumping in this ion.
By finding the proper laser frequency for pumping, the ratio of ions in an excited metastable state to
those in the ground state will be altered. The result should be easily measurable as ions in the
different states exhibit distinct mobilities and thus drift at different speeds through the apparatus
to the detector.
The technique is fast because ions are probed “as is”, as delivered without any further delays or
preparation steps. In addition, broadband initial level searches as well as hyperfine spectroscopy may
be performed utilizing the same setup.
Although we focus on Lr+, the concept offers unparalleled access to laser spectroscopy of many other
mono-atomic ions across the periodic table, in particular, the transition metals including the
high-temperature refractory metals and elements beyond lawrencium.
Other ionic species like triply-charged thorium shall be within reach of the LRC approach as well.
Moreover, the method enables to optimize signal-to-noise ratios and thus ease ion mobility spectrometry,
state-selected ion chemistry and other applications.
Theoretical modelling
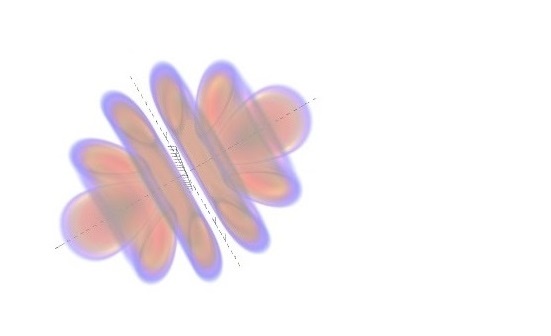
Atomic model calculations guide our experiments by predicting spectral ranges for atomic transitions.
In order to be accurate and reliable, the calculations must be performed in a relativistic framework
and need to treat correlation, i.e., instantaneous interaction between the electrons, on a highest possible
level.
In close collaboration with our external partners, we carry out relativistic Fock space coupled
cluster (FSCC) calculations to predict the dominant spectral lines and their wavelengths in Lr+, Rf+, and
Db+ as well as in their lighter chemical homologues, the spectra of which are known and thus also serve to
validate our calculations. In order to be able to use large model spaces and thus obtain optimal accuracy,
the FSCC method is augmented by the intermediate Hamiltonian scheme. This approach is also used to
predict the hyperfine structure constants and the field shifts of the lowest excited states of the ions.
In addition, we apply the relativistic configuration interaction methods to deliver reliable
predictions of the lifetimes and branching fractions of the states of interest. Upon these numbers, we can
better estimate the signal-to-noise ratios in the envisaged experiments.
Moreover, we develop ab-initio coupled cluster methods for predicting the transport properties of the ions
in noble gases. These latter methods elucidate (from the theory point of view) the trends in ion-atom
interactions and the dependence of these interaction features on the configuration of the ionic states.
Our studies enable a prediction of the optimal working parameters, e.g., best chromatography
performance, for the LRC measurements.
